Creating Synapses and Supporting Neural Cells
- Reviewed16 Jan 2023
- Author Lindzi Wessel
- Source BrainFacts/SfN
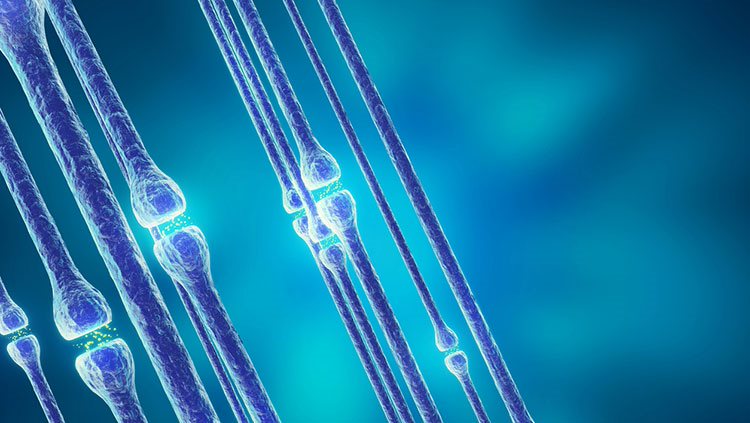
After neurons migrate and settle into their home locations, they begin to form connections with each other. This specialized connection between neurons is called a synapse. At the synapse, only a tiny space separates the signaling portion of the axon from the receiving portion of the dendrite.
Electrical signals that travel down the axon trigger the release of chemical messages called neurotransmitters, which diffuse across this space and are received by receptors on the target dendrite. Such chemical cues can either promote or hinder the generation of a new electrical signal in the receiving neuron. The combined effects of such cues from thousands of synapses ultimately determine how a receiving neuron responds. A human brain contains trillions of these synapses, which gives rise to the brain’s astounding capacity for information processing.
For this processing to occur properly, the formation of synaptic connections must be highly specific. Some specificity is the result of the mechanisms that guide each axon to its proper target. Additional molecules mediate target recognition when the axon reaches the proper location.
Dendrites are also actively involved in initiating contact with axons, and both sides produce proteins that span the space between them and anchor the synapse together.
Once initial contact is established, a synapse continues to differentiate. On the presynaptic side, the tiny axon terminal that contacts the dendrite becomes specialized for releasing neurotransmitters, stocking itself with neurotransmitter packets and proteins that enable those packets to be held in place and then released. On the dendritic — or postsynaptic — side, receptors that respond to those neurotransmitters begin to dot the membrane. Both processes ensure that a synapse can transmit signals quickly and effectively.
New evidence has implicated a third important player in the proper formation of a synapse. Astrocytes are a type of glial cell in the brain previously thought to simply provide scaffolding and passive support to neurons. They are now known to exert their own influence on synaptic development and function.
Many synapses in the brain are contacted by astrocytes, and studies in rodents have found that a single astrocyte can contact thousands of synapses across multiple neurons. The importance of astrocytes in synapse formation is also shown in other studies. Some neurons form only a few synapses when developing in a culture dish from which astrocytes are absent, and recent research has discovered that molecules secreted by astrocytes regulate aspects of synaptic development.
Scientists are learning that molecules from multiple sources work together to promote proper synapse formation. It is now thought that defects in such molecules could contribute to disorders such as autism. In addition, the loss of certain other molecules might underlie the degradation of synapses that occurs during aging.
An array of signals determines which type of neurotransmitter a neuron will use to communicate. For some cells, such as motor neurons, the type of neurotransmitter is fixed (acetylcholine), but for other neurons, it is not. Scientists have found that when certain immature neurons are maintained in a culture dish with no other cell types, they produce the neurotransmitter norepinephrine. In contrast, when the same neurons are cultured with specific cells, such as cardiac tissue, they produce the neurotransmitter acetylcholine.
Just as genetic and environmental signals can modulate the development of specialized cells, a similar process leads to production of specific neurotransmitters. Many researchers believe that the signal to engage the gene, and therefore the final determination of the chemical messenger a neuron will produce, is influenced by factors that come from the location of the synapse itself.
Insulation that covers wires preserves the strength of electrical signals that travel through them. The myelin sheath that covers axons serves a similar function. Myelination, the fatty wrapping of axons by extensions of glia, increases — by as much as 100 times — the speed at which signals can travel along axons. This increase is a function of how the sheath is wrapped, with somewhat regularly spaced gaps called nodes of Ranvier interrupting the sheath. The alternating pattern of insulation and nodes allows electrical signals to move down an axon faster, jumping from one node to the next. This phenomenon, called saltatory conduction (“saltatory” means “leaping”), is responsible for more rapid transmission of electrical signals. Formation of myelin occurs throughout the lifespan.
Adapted from the 8th edition of Brain Facts by Lindzi Wessel.
CONTENT PROVIDED BY
BrainFacts/SfN
References
Clarke, L. E., & Barres, B. A. (2013). Emerging roles of astrocytes in neural circuit development. Nature Reviews Neuroscience, 14(5), 311-321. doi:10.1038/nrn3484
Dabrowski, A., Johnson-Venkatesh, E., & Umemori, H. (2017). Synaptic Development. Conns Translational Neuroscience, 89-111. doi:10.1016/b978-0-12-802381-5.00006-3
Ganguly, K., & Poo, M. (2013). Activity-Dependent Neural Plasticity from Bench to Bedside. Neuron, 80(3), 729-741. doi:10.1016/j.neuron.2013.10.028
Homem, C. C., Repic, M., & Knoblich, J. A. (2015). Proliferation control in neural stem and progenitor cells. Nature Reviews Neuroscience, 16(11), 647-659. doi:10.1038/nrn4021
Kolb, B., & Gibb, R. (2014). Searching for the principles of brain plasticity and behavior. Cortex, 58, 251-260. doi:10.1016/j.cortex.2013.11.012
Lenroot, R. K., & Giedd, J. N. (2006). Brain development in children and adolescents: Insights from anatomical magnetic resonance imaging. Neuroscience & Biobehavioral Reviews, 30(6), 718-729. doi:10.1016/j.neubiorev.2006.06.001
Noctor, S., Cunningham, C., & Kriegstein, A. (2013). Radial Migration in the Developing Cerebral Cortex. Cellular Migration and Formation of Neuronal Connections, 299-316. doi:10.1016/b978-0-12-397266-8.00027-2
Ozair, M. Z., Kintner, C., & Brivanlou, A. H. (2012). Neural induction and early patterning in vertebrates. Wiley Interdisciplinary Reviews: Developmental Biology, 2(4), 479-498. doi:10.1002/wdev.90
Perrimon, N., Pitsouli, C., & Shilo, B. (2012). Signaling Mechanisms Controlling Cell Fate and Embryonic Patterning. Cold Spring Harbor Perspectives in Biology,4(8). doi:10.1101/cshperspect.a005975
Plasticity. (2012, April 1). Brainfacts.org. Retrieved from: http://www.brainfacts.org/brain-basics/brain-development/articles/2012/plasticity/
Pollard, T. (2015). New Light on Growth Cone Navigation. Developmental Cell, 35(6), 672-673. doi:10.1016/j.devcel.2015.12.002
Sala, C., & Segal, M. (2014). Dendritic Spines: The Locus of Structural and Functional Plasticity. Physiological Reviews, 94(1), 141-188. doi:10.1152/physrev.00012.2013
Sorg, B. A., Berretta, S., Blacktop, J. M., Fawcett, J. W., Kitagawa, H., Kwok, J. C., & Miquel, M. (2016). Casting a Wide Net: Role of Perineuronal Nets in Neural Plasticity. The Journal of Neuroscience, 36(45), 11459-11468. doi:10.1523/jneurosci.2351-16.2016
Sun, G. J., Zhou, Y., Stadel, R. P., Moss, J., Yong, J. H., Ito, S., Kawasaki, N. K., Phan, A. T., Oh, J. H., Modak, N., et al. (2015). Tangential migration of neuronal precursors of glutamatergic neurons in the adult mammalian brain. Proc. Natl. Acad. Sci., 112, 9484–9489. doi:10.1073/pnas.1508545112
Werker, J. F., & Hensch, T. K. (2015). Critical Periods in Speech Perception: New Directions. Annual Review of Psychology, 66(1), 173-196. doi:10.1146/annurev-psych-010814-015104
What to Read Next
Also In Brain Development
Trending
Popular articles on BrainFacts.org